Explore the fascinating world where sound modulation meets black hole physics, revealing how scientists are simulating and studying these cosmic wonders in laboratory settings.

Sept. 27, 2024 Matthew Pierce
The Science Behind Acoustic Wave Modulation in Dense Fluids
Acoustic wave modulation in dense fluids is a fascinating branch of physics that deals with the manipulation of sound waves in high-density environments. By carefully controlling these waves, scientists can create conditions that mimic the extreme environments around black holes. This technique relies on the principles of fluid dynamics and wave mechanics to achieve the desired effects.
In practice, modulating acoustic waves in a dense fluid involves using specialized equipment to generate and control sound waves with high precision. These waves can interact with the fluid in complex ways, creating patterns and phenomena that are analogous to those found in the vicinity of black holes. This capability opens up new avenues for experimental physics, allowing researchers to study black hole-like conditions in a controlled laboratory setting.
Simulating Black Hole Phenomena in the Lab
One of the most intriguing applications of acoustic wave modulation yet, has to be the simulation of black hole phenomena in a laboratory environment. By modulating acoustic waves in a dense fluid, researchers have been able to create a black hole analog that bends light in ways similar to a real black hole's gravitational pull. This innovative experiment involves carefully controlling the properties of the fluid and the acoustic waves to mimic the intense gravitational effects of a black hole. As the acoustic waves propagate through the fluid, they create regions of varying density that can trap and bend light, much like the way a black hole's immense gravity warps spacetime and captures light.
These acoustic black holes are in fact created by meticulous processes involving the manipulation of charged rubidium atoms. Using configurations that manipulate sound waves, causing them to spiral inward and become confined in a specific region. First, these atoms are trapped within a precisely controlled magnetic field, which confines them to a specific region. Then, a laser is employed to create what's called an electric potential well, a kind of energy landscape that influences the movement of the atoms. This sophisticated setup causes the rubidium atoms to accelerate to speeds exceeding that of sound, thereby generating a supersonic flow. This supersonic flow acts as a barrier, effectively trapping sound waves within it, much like how the immense gravitational pull of a black hole traps light.
This achievement was measured against the Schwarzschild metric, a solution to Einstein's field equations that describes the gravitational field outside a spherical mass, and the results matched perfectly. The ability to replicate such a complex and extreme environment within the confines of a laboratory is a monumental step forward in astrophysics and experimental physics. Allowing scientists to study the bending of light and other phenomena associated with black holes without needing to venture into space. These lab-created black holes provide a valuable tool for testing theories of general relativity and understanding the behavior of matter and light under extreme conditions. Researchers can now observe how light behaves when it encounters the intense gravitational fields similar to those near actual black holes, offering insights into the warping of spacetime and the effects of gravitational lensing. Helping in understanding the interactions between black holes and their surrounding environments, such as the accretion of matter and the emission of high-energy radiation. This controlled setting enables repeated experiments, ensuring that findings are robust and reproducible, thereby significantly advancing our comprehension of black holes in real time.
Translating Black Hole Pressure Waves into Sound
Called Sonification, this fascinating aspect of black hole research involves translating the pressure waves generated by black holes into audible sounds, providing a unique auditory perspective on these cosmic phenomena. Black holes, such as the one at the center of the Perseus galaxy cluster, produce pressure waves that ripple through the surrounding hot gas, creating disturbances that propagate outward. These ripples can be converted into sound waves, although the frequencies they produce are typically far beyond the range of human hearing, often spanning millions of years per oscillation, about 57 octaves below middle C. . By employing advanced data processing techniques, astronomers can shift these frequencies into the audible range, allowing us to 'hear' the otherwise imperceptible symphony of the universe. This process not only makes the study of black holes more accessible but also offers a novel way to analyze the dynamics and interactions within these extreme environments, shedding light on the intricate dance of matter and energy around these enigmatic objects.
Achievements In Understanding Acoustic Black Holes
Physicists have also made significant strides in creating and understanding these acoustic black holes. Not only providing insights into the behavior of sound in extreme conditions but also offering potential applications in areas such as soundproofing, acoustic cloaking, and even quantum computing. For instance, in the field of soundproofing, the principles derived from acoustic black holes can be used to develop materials and structures that can absorb and dissipate sound waves more efficiently, leading to quieter environments in both residential and industrial settings. This could revolutionize the way we design buildings, making homes, offices, and factories significantly quieter and more comfortable. Imagine living in a bustling city but having a home that is a serene oasis, free from the constant noise of traffic and construction. In industrial settings, quieter machinery could lead to safer and more pleasant working conditions, reducing noise pollution and its associated health risks.
In the realm of acoustic cloaking, these advancements could pave the way for technologies that render objects acoustically invisible, which could have significant implications for military and surveillance applications. This means that submarines, drones, and other vehicles could move undetected by sonar, giving a strategic advantage in defense operations. Additionally, this technology could be used in secure communications, ensuring that sensitive information is transmitted without the risk of eavesdropping. The potential for acoustic cloaking extends to everyday life as well, where it could be used to create private spaces in public areas, allowing for confidential conversations in open environments.
Not surprisingly, the study of acoustic black holes also intersects with the cutting-edge field of quantum computing, where understanding the manipulation of sound at quantum levels could lead to breakthroughs in how information is processed and stored, potentially revolutionizing the technology landscape. Quantum computing promises to solve complex problems at unprecedented speeds, and integrating acoustic principles could enhance these capabilities even further. Leading to advancements in fields such as cryptography, artificial intelligence, and big data analysis, transforming industries and driving innovation. The ability to control sound at the quantum level might also open new avenues in medical technology, such as more precise imaging techniques or targeted therapies that use sound waves to treat diseases. The implications of these advancements are vast, promising a future where sound and quantum mechanics work hand in hand to push the boundaries of what is technologically possible.
By studying these acoustic analogs, scientists have explored the very principles of black hole physics, leading to a deeper understanding of both acoustics and general relativity. As well, the creation of these acoustic black holes not just helps researchers understand the behavior of actual black holes on a more accessible scale, but directly observing how sound waves interact with these acoustic black holes, scientists can draw parallels to how light and other forms of radiation interact with them.
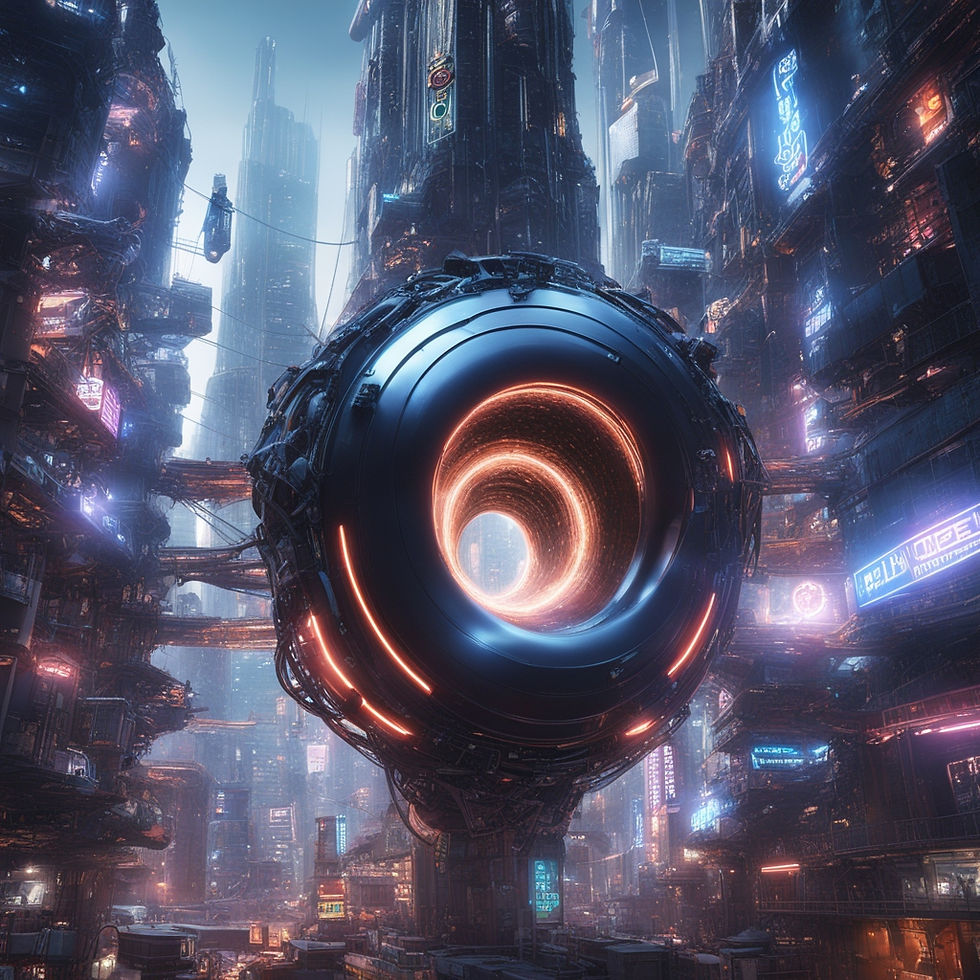
Listening to the Cosmic Dance: The Sound of Black Holes Merging
Another exciting development in astrophysics is the ability to 'listen' to the merger of black holes. These gravitational waves were first predicted by Albert Einstein in his theory of general relativity, but it wasn't until the advent of highly sensitive instruments that we could actually detect them. When two black holes spiral towards each other in a cosmic dance and eventually merge, they produce a distinctive 'chirp'—a rapid increase in frequency and amplitude—that can be detected by sophisticated observatories such as LIGO (Laser Interferometer Gravitational-Wave Observatory) and Virgo. These instruments are capable of measuring incredibly minute distortions in spacetime, allowing scientists to capture the fleeting signals of these cataclysmic events, as well as other celestial bodies. The detection of these 'chirps' not only confirms the existence of gravitational waves but also provides invaluable data about the properties of black holes, such as their masses and spin rates, offering a new way to view, study, and 'hear' the universe.
Essentially, these audio representations help to demystify the complex and often abstract phenomena associated with black holes, bringing the wonders of the universe closer to our everyday experience. We can now envision the universe as a vast orchestral theatre, with every celestial object, including our own, producing its own harmonies and melodies, contributing to an even grander symphony. An ever-changing and expanding sonnet we call reality.
Comments